INTRODUCTION
Clostridioides difficile (previously Clostridium difficile) is a gram-positive spore- forming anaerobic Bacillus recognized as a potential enteric pathogen. In fact, C. difficile infection (CDI) is a significant healthcare-associated infection posing a growing threat to public health worldwide; in the US alone, it accounts for more than 450,000 cases and 29,000 deaths annually, costing approximately $5 billion (1, 2). Additionally, the incidence of intestinal disease caused by CDI is rising, and the incidence and mortality rates among older adults aged 65 years and above are rapidly accelerating, with the risk of infection reaching more than 10 times that of other age groups (3). When antibiotics or drugs destroy the intestinal flora, pathogenic C. difficile colonizes the area and secretes toxin A or toxin B, leading to inflammation (4). The more toxins (toxin A [enterotoxin] or toxin B [cytotoxin]) are excreted, the more severe the disease is (5). Toxins A and B damage the intestinal epithelial cells and activate the host’s inflammatory responses. Patients with CDI show clinical manifestations ranging from mild diarrhea to life-threatening conditions, such as pseudomembranous colitis, fulminant colitis, toxic megacolon, shock, and in severe cases, death (6, 7). C. difficile is a common cause of hospital-acquired infection following antibiotic treatment, inducing a heavy burden on most hospitals (8). The incidence and severity of CDI have been increasing, making it a significant burden on healthcare systems. Hospital-acquired CDI increases the financial burden and prolongs the hospital stay, as well as expanding the complication and mortality and worsening the medical burden. Patients with CDI spend more than $24,000, which is higher than that of patients without CDI (2). Common treatment strategies for CDI mainly involve the administration of antibiotics, such as metronidazole, vancomycin, and fidaxomicin (9). However, the emergence of antibiotic-resistant strains of C. difficile limits the effectiveness of these antibiotics (10). These antibiotics can also disrupt the natural gut microbiota, predisposing patients to recurrent CDI and other opportunistic infections (11). Recently, fecal microbiota transplantation (FMT) from healthy donors for CDI remediation has been reported to be effective, thereby gaining considerable attention as a method for treating recurrent CDI (12, 13). However, FMT is an invasive procedure, and its true efficacy remains unclear, with complications such as those in patients with risk factors for aspiration and long-term safety being its major concerns (14). Furthermore, drug resistance, side effects due to antibiotic treatment, and quality control emerge as primary consequences. Therefore, alternative therapeutic approaches that are effective, specific, and safe are urgently needed.
Recently, bacteriophage-derived endolysins have emerged as a potential solution to combat bacterial infections, including CDI (15). Endolysins are enzymes produced by bacteriophages during the final stage of their life cycle and are responsible for breaking down the bacterial cell wall to release progeny phage particles (16). Given their high specificity for the bacterial cell wall, endolysins have garnered attention as alternative antimicrobial agents with minimal impact on the commensal microbiota (17). Several endolysins have been identified and characterized as bacteriophages that infect C. difficile (16, 17, 18, 19, 20).
This study aimed to investigate the potential of the bacteriophage-derived endolysin CHAPSAP26-161 as a therapeutic agent to combat CDI. CHAPSAP26-161 showed a promising bactericidal activity against C. difficile strains. Its efficacy in animal models of CDI was also explored. CHAPSAP26-161 reduced CDI mortality in mice, significantly improving C. difficile-induced systemic inflammation and the damaged intestinal barrier. In addition, treatment with CHAPSAP26-161 at various concentrations remarkably reduced C. difficile burden and clinical symptoms such as diarrhea and weight loss. Thus, this study contributes to the growing body of research exploring the application of bacteriophage-derived endolysins as a potential therapeutic option for CDI.
MATERIALS AND METHODS
Bacterial strains and Culture conditions
We purchased C. difficile ATCC 9689T from ATCC (Manassas, VA, USA), and three clinical isolates of C. difficile (KPN10P03654, KPN10P03780, and KPN10P03783) from the Pathogen Resource Bank of Kyungpook National University Hospital (Daegu, Republic of Korea). The seven strains of C. difficile (CD-H-1, CD-H-2, CD-H-7, CD-M-5, CD-M-7, CD-M-9, and CD-M-11) used in this study were isolated from fecal samples of inflammatory bowel disease patients at Kyungpook National University Hospital in Daegu, Republic of Korea, between 2020 and 2022. All strains were cultured anaerobically inside an anaerobic gas chamber (BACTRON300; Sheldon Manufacturing Inc., Cornelius, OR, USA) at 37°C for 24-48 h in Mueller Hinton broth (MHB; BD, Franklin Lakes, NJ, USA), Mueller Hinton agar (MHA; BD, Franklin Lakes, NJ, USA), Brain Heart Infusion broth (BHI; BD, Franklin Lakes, NJ, USA), Brain Heart Infusion agar (BHA; BD, Franklin Lakes, NJ, USA), and blood agar plates (BAP; Synergy Innovation Co. Ltd., Gyeonggi-Do, Republic of Korea).
Protein expression and Purification
Escherichia coli BL21 (DE3) Star® was transformed with CHAPSAP26-161 (483 bp) plasmids. Transformants were incubated in 1 L of lysogeny broth at 150 rpm and 37°C with ampicillin (150 μg/mL) until culture absorbance reached OD 0.5 at 600 nm. We added isopropyl β-d-1-thiogalactopyranoside to a final concentration of 0.1 mM and incubated the culture solution at 18°C for 16 h. Then, we cooled the culture medium on ice and centrifuged it at 6,000 rpm (at 4°C for 10 min) to obtain the cells. These cells were resuspended in 30 mL of lysis buffer (50 mM Tris-HCl [pH 8.0], 200 mM NaCl, and 100 μM ZnCl2). Ultrasonication was repeated 10 times for 5 min to disrupt the bacterial cells and centrifuged at 15,000 rpm (at 4°C for 30 min). After centrifugation, the supernatant was filtered through a 0.22 μm syringe filter (GVS Abulo; Stanford, ME, USA). The filtered solution was then purified using a 5 mL His-trap column (GE Healthcare; Chicago, IL, USA) installed in a high-speed protein liquid chromatography system (GE AKTA Prime Plus FPLC System; Uppsala, Sweden). Residual imidazole was removed by dialysis against a lysis buffer of Tris-HCl (20 mM, pH 8.0) and NaCl (0.5 M). The purity and size of the protein CHAPSAP26-161 (19.7 kDa) were confirmed by SDS-PAGE, and the protein concentration was measured using a nanodrop spectrophotometer (ND-2000; Thermo Scientific; Waltham, MA, USA). Polyclonal rabbit anti-mouse immunoglobulin G was conjugated with recombinant abysin and horseradish peroxidase, using an anti-His-Tag monoclonal mouse antibody (Ab Frontier, Republic of Korea) as the first antibody and 6X His-tag as a secondary antibody for Western blot analysis.
Minimum inhibitory concentration (MIC) and Minimum bactericidal concentration (MBC)
The MIC and MBC values of CHAPSAP26-161 were determined using a broth microdilution method with a slight modification, as described by the Clinical and Laboratory Standards Institute (21), in round-bottomed 96-well microplates (SPL Life Sciences, Pochan, Gyeonggi-do, Republic of Korea). The exponentially grown bacterial cells were diluted to 105 CFU/mL concentration in MHB and then incubated with CHAPSAP26-161 (1-100 μg/mL) at 37°C for 24 h. We defined MIC as the lowest antibacterial concentration that completely inhibited bacterial growth. To determine the MBC, we spotted 10 μL of the mixture from each 96-well MIC test plate on MHA to evaluate bacterial viability. MBC was defined as the lowest concentration of antimicrobial agent with no growth on the agar plate. All assays were performed thrice. Moreover, we included growth and sterility controls, with LysSAP26 used as a positive control for MIC and MBC determination (22, 23).
C. difficile toxin assay
Analysis of toxins A and B at the DNA level by PCR amplification
DNA samples for PCR amplifications were prepared using the boiling method. Briefly, 1-2 colonies of culture were taken from the agar plate and resuspended in 200 µL of RNase-free water (Welgene Inc., Gyeongsan, Republic of Korea) in a 1.5 mL Eppendorf tube. To settle the bacterial debris, we boiled the suspension was boiled for 7-10 min and centrifuged it at 10,000× g for 5 min. The supernatant containing the genomic DNA was then transferred to clean microcentrifuge tubes and used for PCR amplification. The remaining aliquots were stored at 20°C for future use. We used the tpi-specific primers (tpi-F [5′-AAA GAA GCT ACT AAG GGT ACA AA-3′] and tpi-R [5′-CAT AAT ATT GGG TCT ATT CCT AC-3′]) to detect the product of the 230 bp tpi gene specific to C. difficile. To detect toxins A and B, we incorporated toxin A-specific primer sets (tcdA-F [5′-AGA TTC CTA TAT TTA CAT GAC AAT AT-3′] and tcdA-R [5′-GTA TCA GGC ATA AAG TAA TAT ACT TT-3′]) and toxin B-specific primer sets (tcdB-F [5′-GGA AAA GAG AAT GGT TTT ATT AA-3′] and tcdB-R [5′-ATC TTT AGT TAT AAC TTT GAC ATC TTT-3′]) to amplify the 369 and 160 bp tcdA and tcdB genes, respectively (24). PCR was amplified in 50 μL of combined TaKaRa Ex Taq (0.25 μL [5 U/μL]), 10X Ex PCR buffer (Mg2+ plus, 5 μL), dNTP mixture (4 μL [2.5 mM each]), each primer (0.2 μM), and DNA template (1.5 μL), with the following cycling conditions: 95°C for 3 min; 40 cycles of 30 s at 95°C, 1 min at 50°C, 90 s at 72°C, and 10 min at 68°C. Additionally, genomic DNA was extracted using the QIAamp® DNA Mini Kit (Qiagen; Hilden, Germany) according to the manufacturer’s instructions. We designed the primers to obtain full-length tcdB genes of 7.1 kb and amplified them with forward and reversed primer sets tcdB_start (5′-ATA GTA AAG GAG AAA ATT TTA TG-3′) and tcdB_stop (5′-CTA TTC ACT AAT CAC TAA TTG AGC-3′). PCR was amplified in 50 μL of combined TaKaRa LA Taq (0.5 μL [5 U/μL]), 10X LA PCR buffer (Mg2+ free, 5 μL), MgCl2 (5 μL [final 2.5 mM]), dNTP mixture (8 μL [2.5 mM each]), each primer (0.3 μM), and DNA template (2 μL), with the following cycling conditions: 94°C for 1 min, 32 cycles of 20 s at 98°C, 30 s at 47°C, 8 min at 68°C, and 10 min at 72°C. Similarly, to further confirm the tcdA gene, we designed the tcdA-specific forward primer NK3 [5′-GGA AGA AAA GAA CTT CTG GCT CAC TCA GGT-3′] and reverse primer NK2 [NK2: 5′-ACC AAT AGA AGA TTC AAT ATT AAG CTT-3′] to amplify a 252 bp fragment of the tcdA gene under the following thermocycler conditions: 94°C for 1 min, followed by 30 cycles of 20 s at 98°C, 30 s at 50°C, 1 min at 68°C, and 10 min at 72°C. All oligonucleotides were synthesized by Macrogen (Seoul, Republic of Korea). PCR amplifications were visualized by Gel Documentation System (Atto; Tokyo, Japan) after final electrophoresis on a 1% agarose gel stained with EcoDye (Solgent; Daejeon, Republic of Korea).
Analysis of toxins A and B at the protein level
Toxins A and B from 11 C. difficile strains were identified by sandwich enzyme-linked immunosorbent assay (ELISA). In particular, we detected toxin A by using the EDITM Fecal C. difficile Toxin A ELISA Kit (Epitope Diagnostic Inc.; San Diego, CA, USA), and toxin B by using the EpilisaTM Fecal C. difficile Toxin B ELISA Kit (Epitope Diagnostic Inc.; San Diego, CA, USA). All results were analyzed and interpreted according to the manufacturer’s instructions.
Cytotoxicity assays
The A549 cell line’s (Sigma-Aldrich; St. Louis, MO, USA) viability was measured by 3-(4,5-methylthiazol-2-yl)-2,5-diphenyl- tetrazolium bromide (MTT) assay using an MTT cell viability assay kit (Amresco Inc.; Solon, OH, USA) according to the manufacturer’s instructions. Briefly, A549 cells (1 × 105 cells/well) were seeded in a 24-well plate containing RPMI 1640 medium (Sigma-Aldrich; St. Louis, MO, USA) and kept overnight at 37°C in a CO2 incubator for attachment. The next day, we replaced the medium with fresh RPMI 1640 medium with various CHAPSAP26-161 concentrations (25-1,000 μg/mL, RPMI 1640 medium/well) and allowed the cells to grow for 24 h. After removing the medium, we washed the cells with phosphate-buffered saline (PBS), added 250 μL of MTT solution (0.5 mg/mL) to each well, further incubated the cells for 2 h, and then added 250 μL of solubilizing solution (90% isopropanol, 0.01% Triton X-100, and 0.01N HC5l79l). Color development after the reaction was measured at 570 nm using a VersaMax™ microplate reader (Molecular Devices, San Jose, CA, USA). Each experiment was performed in triplicate.
Experimental design of endolysin therapy in an acute CDI murine model
Female neutropenic C57BL/6 mice (5 weeks old, 16-19 g; Orient Bio, Seongnam, Gyeonggi-do, Republic of Korea) were housed (5 mice/cage) under controlled conditions (50% humidity and a 12 h:12 h light:dark cycle; specific pathogen-free) and fed a rat/mouse 18% 5L79 diet (PMI Nutrition International, Brentwood, MO, USA). Three different strains of C. difficile (C. difficile ATCC 9689T, CD-M-5, and CD-H-7) were used for CDI. An antibiotic mixture of kanamycin (0.4 mg/mL; Sigma-Aldrich, USA), gentamicin (0.035 mg/mL; Sigma-Aldrich; St. Louis, MO, USA), colistin (850 U/mL; Sigma-Aldrich; St. Louis, MO, USA), metronidazole (0.215 mg/mL; Sigma-Aldrich, USA), and vancomycin (0.045 mg/mL; Sigma-Aldrich; St. Louis, MO, USA) was prepared in the mice’s drinking water. We deployed a uniform CDI murine model with a modification, as described by Chen et al. (25) and De Wolfe et al. (1). Briefly, mice were randomly grouped into healthy control (HC), CHAPSAP26-161 control, CDI control, and CHAPSAP26-161-treated CDI groups. CDI control or CHAPSAP26-161-treated mice were allowed to drink the antibiotic cocktail for 5 days followed by sterile drinking water for 2 days. Mice received a single dose of clindamycin (20 mg/kg, Sigma-Aldrich; St. Louis, MO, USA) one day before C. difficile inoculation. On day 1, mice were challenged with 200 μL of C. difficile cells (5.0 × 109 CFU) via oral gavage. After 3 h of C. difficile challenge, they received 150-300 μg of CHAPSAP26-161 endolysin in 200 μL of Dulbecco’s phosphate-buffered saline (1X D-PBS, Welgene, Republic of Korea) intrarectally. All mice were monitored for 7 days. On day 8, they were given oral gavage of Clostridioides (5.0 × 109 CFU) again in the same way as mentioned earlier, and after 3 h, they received CHAPSAP26-161 endolysin intraperitoneally in the same amount (200 μL). Fig. 1 provides a graphical representation of the CDI model. The mice were divided into 14 different groups as described below. They were observed for disease signs, such as diarrhea, hunched posture, wet tail, and weight loss. The diarrhea was graded as 0, 1, and 2 for normal, loose, and liquid stools or soiled tail, respectively, as described by Tam et al. (26). In addition, we recorded their symptoms, survival, and weight daily. All mice were monitored for 14 days after the C. difficile challenge and euthanized by CO2 asphyxiation using dry ice according to the American Veterinary Medical Association guidelines (https://www.avma.org/sites/default/files/2020-02/Guidelines-on- Euthanasia-2020.pdf). Fecal, colon, and tissue samples from the cecum and large intestines were collected for histopathological analysis. Moreover, we observed the mice daily for mortality and morbidity; those that were judged to be in a moribund state were euthanized, and their fecal, colon, and tissue samples were collected for histopathological examination.
The mice were divided into 14 groups as follows:
Group 1. The HC group was injected with 200 μL of the PBS alone (5 mice/group).
Group 2. The first CHAPSAP26-161 control group was injected with 150 μg of CHAPSAP26-161 alone (5 mice/group).
Group 3. The second CHAPSAP26-161 control group was injected with 300 μg of CHAPSAP26-161 alone (5 mice/group).
Group 4. The third CHAPSAP26-161 control group was injected with 500 μg of CHAPSAP26-161 alone (5 mice/group).
Group 5. Negative control group receiving antibiotics was injected with only 100 μL of PBS (5 mice/group).
Group 6. First CDI control group infected with C. difficile ATCC 9689T (4 mice/group).
Group 7. First endolysin-treated group infected with C. difficile ATCC 9689T was treated with 150 μg of CHAPSAP26-161 after 3 h of C. difficile challenge (4 mice/group).
Group 8. First endolysin-treated group infected with C. difficile ATCC 9689T was treated with 300 μg of CHAPSAP26-161 after 3 h of C. difficile challenge (4 mice/group).
Group 9. Second CDI control group infected with CD-M-5 (3 mice/group).
Group 10. Second endolysin-treated group infected with CD-M-5 was treated with 150 μg of CHAPSAP26-161 after 3 h of C. difficile challenge (3 mice/group).
Group 11. Second endolysin-treated group infected with CD-M-5 was treated with 300 μg of CHAPSAP26-161 after 3 h of C. difficile challenge (3 mice/group).
Group 12. Third CDI control group infected with Clostridioides CD-H-7 (3 mice/group).
Group 13. Third endolysin-treated group infected with CD-H-7 was treated with 150 μg of CHAPSAP26-161 after 3 h of C. difficile challenge (3 mice/group).
Group 14. The third endolysin-treated group was infected with CD-H-7 and treated with 300 μg of CHAPSAP26-161 after 3 h of C. difficile challenge (3 mice/group).
The Animal Care Committee of Kyungpook National University (Daegu, Republic of Korea) approved this experiment (2022-0339-2).
Histopathological analysis
Histopathological analyses were conducted to evaluate the inflammation and mucosal damage of the colon or cecum tissues caused by CDI. Cecum or colon tissue samples were fixed in 5% paraformaldehyde, embedded in paraffin, and sliced into 3 µm-thick sections. The deparaffinized sections were then stained with hematoxylin and eosin (H&E). We also stained them with periodic acid-Schiff (PAS) by using the PAS Stain Kit (Mucin Stain) (ab150680) (Abcam; Cambridge, United Kingdom) according to the manufacturer instructions. Using an Olympus SZX12 microscope (Tokyo, Japan), we observed and captured images of the tissue sections.
Microbiome analysis in murine feces
Bacterial DNA was extracted from fecal samples collected from euthanized mice, using a FastDNA® spin kit for soil (MP Biomedicals, CA, USA) according to the manufacturer’s instructions. The 16S rRNA genes were amplified using the universal primer pairs 338F (5′-ACT CCT ACG GGA GGC AGC AG-3′) and 806R (5′-GGA CTA CHV GGG TWT CTA AT-3′) corresponding to the V3-V4 region. These genes were sequenced using the Illumina MiSeqTM PE300 platform at Accugene Inc. (Incheon, Republic of Korea). Briefly, the sequence data were processed with QIIME 2 (27). We filtered raw sequence data (FATSQ) and then removed chimeras by using CD-HIT-OTU and rDNA tools (28, 29). Subsequently, the assembled reads were clustered into operational taxonomic units (OTUs) using the UPARSE-OTU algorithm at a cutoff value of 97% identity at the species level (30). All representative sequences from each OTU were used for taxonomic assignment, and phylum-to-species level characterization was conducted using the Silva database (https://www.arb-silva.de). Alpha- diversity metrics (Shannon, Simpson, Chao 1, observed features, and Faith’s phylogenetic diversity (31)), beta-diversity metrics (weighted UniFrac, unweighted UniFrac, Jaccard distance, and Bray-Curtis dissimilarity), and principal coordinate analysis were estimated using q2-diversity. The differences between groups in taxonomic composition taxa were analyzed using the linear discriminant analysis (LDA) effect size (LEfSe) analysis, and LDA scores greater than 4 indicated discriminative taxa. Raw sequencing data have been uploaded to the NCBI Sequence Read Archive (SRA) database under BioProject ID: PRJNA1070869.
Statistical analysis
All statistical data were analyzed using OriginPro 2023b (OriginLab Corporation, Northampton, MA, USA). Kaplan-Meier survival analysis was used for the log-rank (Mantel-Cox) test. Statistical significance was measured by one-way ANOVA followed by Tukey’s test or Kruskal-Wallis test. Correlations between relevant variables were analyzed using Spearman’s rank correlation test. A P-value less than 0.05 was considered statistically significant.
RESULTS
Endolysin CHAPSAP26-161 purification
The CHAP SAP26-161 gene was successfully cloned into the pET-21a (+) vector and expressed in E. coli cells bearing pET21a_CHAPSAP26-161. The purified protein demonstrated the correct mass and near homogeneity with LysSAP26 (29.1 kDa) and CHAPSAP26-161 (19.7 kDa) by SDS-PAGE analysis. The protein concentrations were 3.39 and 23.81 mg/L for LysSAP26 and CHAP SAP26-161, respectively (32).
MIC and MBC
The MIC and MBC values of CHAPSAP26-161 against 11 strains of C. difficile were 50 µg/mL (Table 1). In all cases, these values had equal concentrations of LysSAP26 (75 µg/mL) and CHAPSAP26-161 (50 µg/mL).
Table 1.
MIC and MBC of endolysins LysSAP26 and CHAPSAP26-161 against 11 strains of C. difficile
SN | Strains | LysSAP26 | CHAPSAP26-161 | ||
---|---|---|---|---|---|
MIC | MBC | MIC | MBC | ||
1 | C. difficile CD-H-1 | 75 | 75 | 50 | 50 |
2 | C. difficile CD-H-2 | 75 | 75 | 50 | 50 |
3 | C. difficile CD-H-7 | 75 | 75 | 50 | 50 |
4 | C. difficile CD-M-5 | 75 | 75 | 50 | 50 |
5 | C. difficile CD-M-9 | 75 | 75 | 50 | 50 |
6 | C. difficile CD-M-9 | 75 | 75 | 50 | 50 |
7 | C. difficile CD-M-11 | 75 | 75 | 50 | 50 |
8 | C. difficile ATCC 9689T | 75 | 75 | 50 | 50 |
9 | C. difficile KPN10P03654 | 75 | 75 | 50 | 50 |
10 | C. difficile KPN10P03780 | 75 | 75 | 50 | 50 |
11 | C. difficile KPN10P03783 | 75 | 75 | 50 | 50 |
Toxigenic and nontoxigenic isolates
The species-specific internal fragment of the tpi gene was detected in the 11 strains of Clostridioides. Of the 11 isolates, eight toxigenic isolates (A+B+) showed tpi, tcdA, and tcdB amplification signals (in multiplex PCR as well), whereas three nontoxigenic isolates (A−B−) showed only tpi amplification signals using the primer sets tpi-F and tpi-R; tcdA-F and tcdA-R; and tcdB-F and tcdB-R. Interestingly, in immunogenic assays using ELISA kits, one isolate (CD-M-5) was found to be nontoxigenic (Table 2). Furthermore, we amplified the full size (7.1 kb) of the tcdB gene using primer sets tcdB_start and tcdB_stop and another flanking region of the tcdA gene using primer sets NK3 and NK2 to confirm the toxinotype. Finally, strain CD-M-5 was found to be nontoxigenic (A−B−).
Table 2.
Analysis of the toxigenic and nontoxigenic strains of C. difficile according to genetic and protein levels.
SN | Strains | C. difficile Toxins | |||||
---|---|---|---|---|---|---|---|
Genetic level | Protein level | ||||||
Toxin A (tcdA) | Toxin B (tcdB) | Toxin A | Toxin B | Concentrations (mg/mL) | |||
Toxin A | Toxin B | ||||||
1 | C. difficile CD-H-1 | + | + | + | + | 3.2966 | 1.1933 |
2 | C. difficile CD-H-2 | - | - | - | - | 0.0516 | 0.06835 |
3 | C. difficile CD-H-7 | + | + | + | + | 3.2299 | 1.23045 |
4 | C. difficile CD-M-5 | - | - | - | - | 0.0647 | 0.0641 |
5 | C. difficile CD-M-7 | + | + | + | + | 3.3323 | 1.2546 |
6 | C. difficile CD-M-9 | - | - | - | - | 0.066 | 0.06675 |
7 | C. difficile CD-M-11 | - | - | - | - | 0.0655 | 0.0688 |
8 | C. difficile ATCC 9689T | + | + | + | + | 3.5496 | 2.2512 |
9 | C. difficile KPN10P03654 | + | + | + | + | 2.71365 | 1.2107 |
10 | C. difficile KPN10P03780 | + | + | + | + | 3.02125 | 1.215 |
11 | C. difficile KPN10P03783 | + | + | + | + | 2.97335 | 1.352 |
Cytotoxicity of CHAPSAP26-161
A549 cells confirmed CHAPSAP26-161’s cytotoxic ability in eukaryotic cells at specific concentrations, considering that epithelial cells represent the first line of defense against bacteria or bacterial products. The cell toxicity of the endolysin CHAPSAP26-161 was evaluated on A549 human adenocarcinoma alveolar basal epithelial cells by MTT assay dose-dependently. CHAPSAP26-161 showed no cytotoxic effect on the A549 cell line at 750 µg/mL or under, where the cell viability was more than 85% (cell viability > 80% are considered noncytotoxic) (33), indicating CHAPSAP26-161 as a safe therapeutic agent. However, at 1,000 µg/mL, the cell viability was 74.8% ± 0.37%.
Endolysin therapy reduces CDI-induced clinical outcomes and colon injury in mice
Change in body weight indicates the general health of mice during CDI. Body weight loss in CDI-induced mice is a marker of disease progression and represents CDI severity (25). All mice from the CDI group, which was infected with C. difficile spores of the strains ATCC 9689T, CD-H-7, and CD-M-5, lost body weight compared with the HC group (Fig. 2a). Furthermore, all those from the HC group, negative control group, and second CDI control group infected with C. difficile CD-M-5 (A−B−) survived (Fig. 2b). Conversely, all mice from the first CDI control group infected with C. difficile ATCC 9689T (A+B+) and the third CDI control group infected with CD-H-7 (A+B+) died after 8 (2 mice by day 6 and 2 mice by day 8) and 9 (one mouse by day six and two mice by day nine) days of postinfection, respectively (Fig. 2b). Importantly, all mice from the endolysin (CHAPSAP26-161)-treated control groups infected with the C. difficile ATCC 9689T and CD-H-7 survived (Fig. 2b). Oral gavage of CHAPSAP26-161 (at a different concentration, 150 µg/mL and/or 300 µg/mL) improved not only body weight loss after infection but also diarrhea (Fig. 2a). In addition, CHAPSAP26-161 recovered the colon lengths that were shortened because of CDI (Fig. 3). Immunohistological staining (H&E and PAS) showed that CHAPSAP26-161 administration reduced histological injuries such as histological structure destruction and epithelial damage caused by CDI in the colon (Fig. 4).
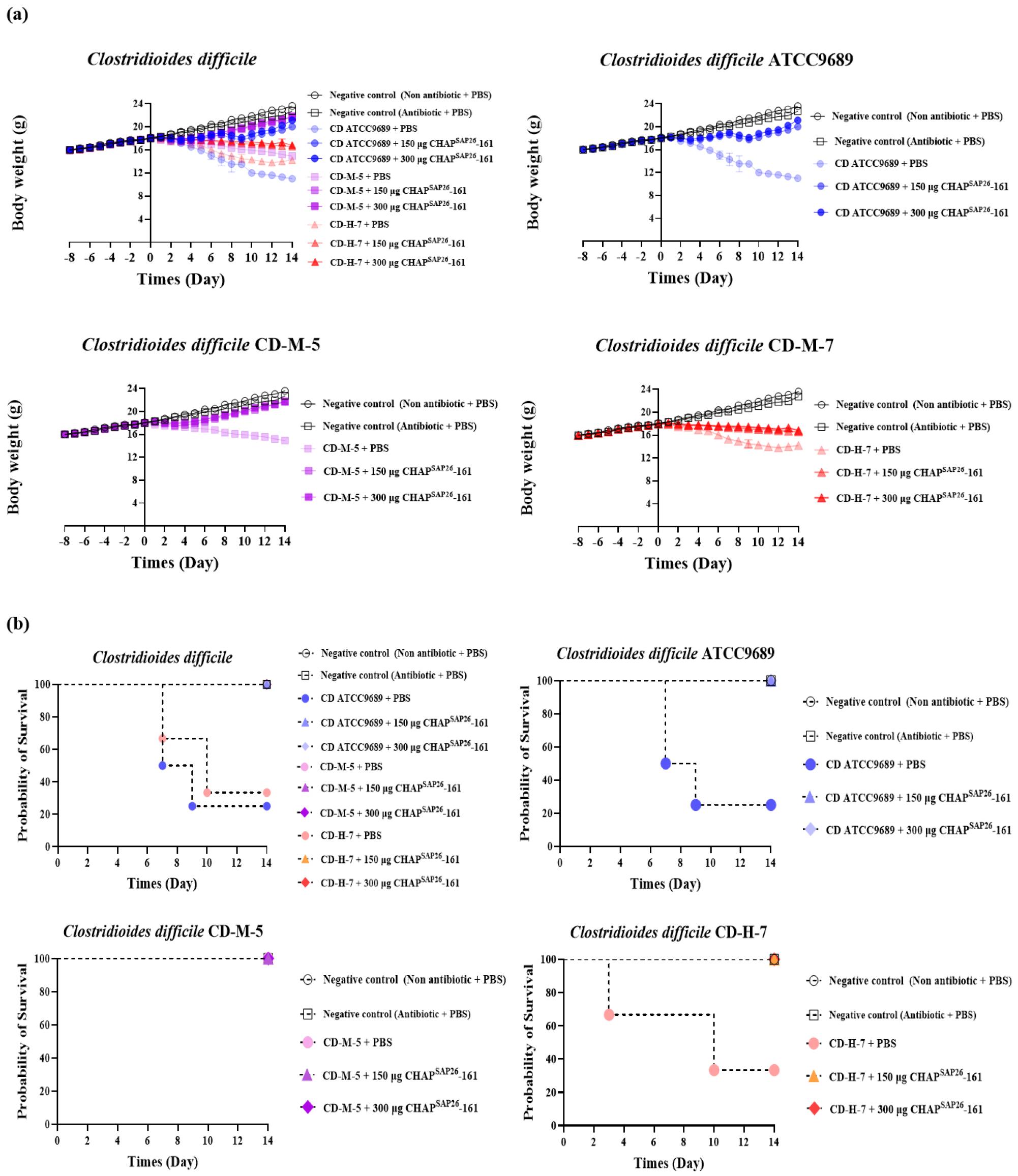
Fig. 2
Clinical outcomes of mice with CDI. Body weight variation (A) and Kalpan-Meier survival curve (B) of healthy control, negative control, CDI control, and endolysin-treated groups. CDI, C. difficile infection.
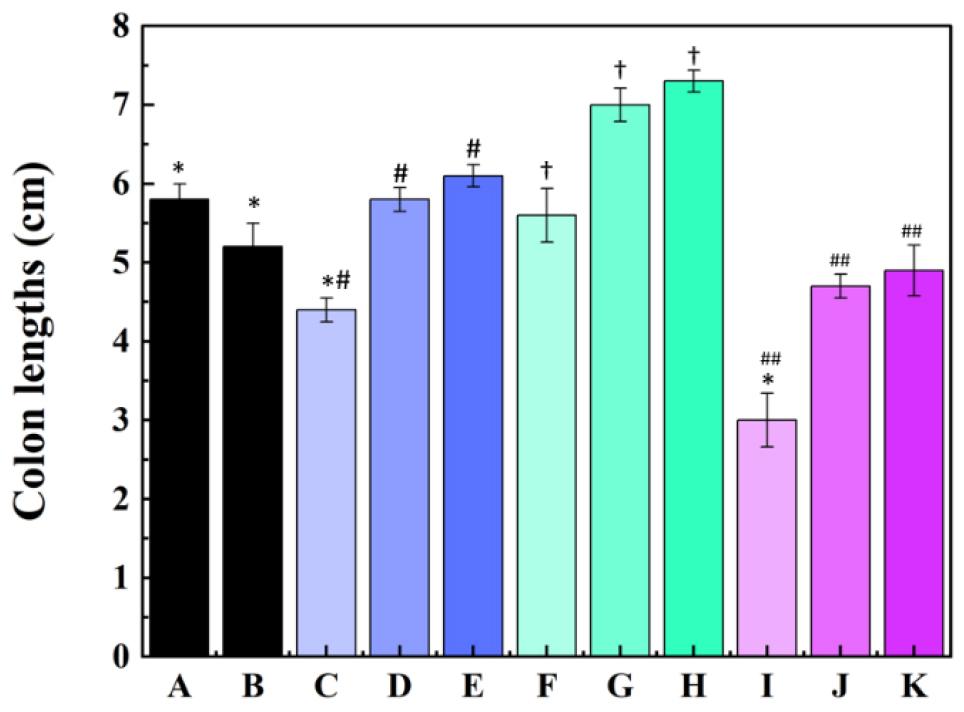
Fig. 3
Colon length after CHAPSAP26-161 treatment. (A) Healthy control group; (B) negative control group; (C) first CDI control group (C. difficile ATCC 9689 + PBS); (D) first endolysin-treated group (C. difficile ATCC 9689 + CHAPSAP26-161 150 µg); (E) first endolysin-treated group (C. difficile ATCC 9689 + CHAPSAP26-161 300 µg); (F) second CDI control group (C. difficile CD-M-5 + PBS); (G) second endolysin-treated group (C. difficile CD-M-5 + CHAPSAP26-161 150 µg); (H) second endolysin- treated group (C. difficile CD-M-5 + CHAPSAP26-161 300 µg); (I) third CDI control group (CD-H-7 + PBS); (J) third endolysin-treated group (C. difficile CD-H-7 + CHAPSAP26-161 150 µg); (K) third endolysin-treated group (C.CDI, C. difficile infection. Statistical significance was indicated: *P < 0.05, #P < 0.001, †P < 0.01, and P < 0.001.
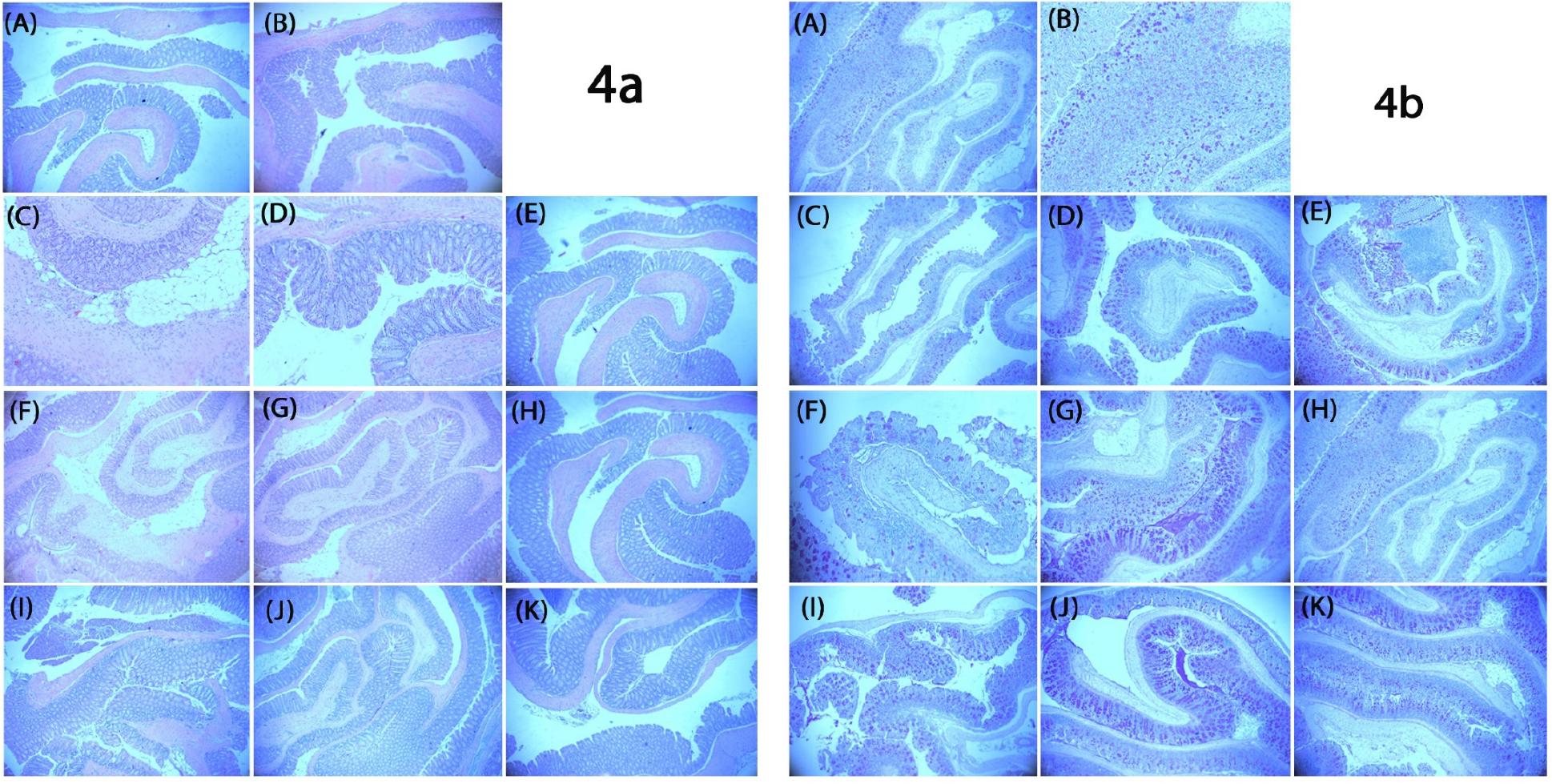
Fig. 4
Representative histological images of colonic tissues stained with H&E (4A) and PAS (4B). The microscope’s magnification was adjusted to 5-10x for use. (A) Healthy control group; (B) negative control group; (C) first CDI control group (C. difficile ATCC 9689 + PBS); (D) first endolysin-treated group (C. difficile ATCC 9689 + CHAPSAP26-161 150 µg/mL); (E) first endolysin-treated group (C. difficile ATCC 9689 + CHAPSAP26-161 300 µg/mL); (F) second CDI control group (C. difficile CD-M-5 + PBS); (G) second endolysin-treated group (C. difficile CD-M-5 + CHAPSAP26-161 150 µg/mL); (H) second endolysin- treated group (C. difficile CD-M-5 + CHAPSAP26-161 300 µg/mL); (I) third CDI control group (CD-H-7 + PBS); (J) third endolysin-treated group (C. difficile CD-H-7 + CHAPSAP26-161 150 µg/mL); (K) third lysin-treated group (C. difficile CD-H-7 + CHAPSAP26-161 300 µg/mL). H&E, hematoxylin and eosin; PAS, periodic acid-Schiff; CDI, C. difficile infection.
Endolysin therapy suppresses gut dysbiosis caused by CDI
Before endolysin therapy, CDI-induced significant alterations in gut microbiota composition. At the phylum level, the composition of the gut microbiota considerably differed between the CDI, HC, negative control, and endolysin-treated groups. The HC group mainly comprised Bacillota (92.4%), followed by Bacteroidota (5.9%), Verrucomicrobiota (0.5%), and Pseudomonadota (0.1%); the negative control group comprised Bacillota (89.1%), Bacteroidota (2%), Verrucomicrobiota (5.3%), and Pseudomonadota (3.6%); meanwhile, the CDI group showed a drastic reduction in Bacillota (44.3%) and an increase in Bacteroidota (2%), Verrucomicrobiota (5.3%), and Pseudomonadota (3.6%) (Fig. 5a). After endolysin treatment, the proportion of Bacillota increased to 60.5%, whereas those of Bacteroidota (19.7%) and Verrucomicrobiota (8.4%) decreased (Fig. 5a). Interestingly, the proportion of Pseudomonadota slightly increased to 11.2% (Fig. 5). At the family level, the proportions of Enterobacteriaceae (6.1%), Staphylococcaceae (3.2%), Porphyromonadaceae (6.2%), Peptostreptococcaceae (4.7%), Bacteroidaceae (29.2%), Erysipelotrichaceae (2.9%), and Christensenellaceae (0.7%) increased by 2,611-, 1,335-, 83.5-, 60.3-, 9.1-, 6.9-, and 3.2-fold, respectively, in the CDI group compared with those in the HC group (Fig. 5b). In addition, the proportions of 14 species, namely, Anaerostipes caccae, Bacteroides caccae, Blautia hansenii, C. difficile, Clostridium innocuum, Clostridium symbiosum, Enterocloster clostridioformis, E. coli, Limosilactobacillus reuteri, Mammaliicoccus sciuri, Parabacteroides goldsteinii, Phocaeicola vulgatus, Providencia alcalifaciens, and Thomasclavelia ramosa increased highly in the CDI group compared with those in the HC group (Fig. 5c). Importantly, the relative proportions of all these 14 species decreased in the endolysin treatment groups compared with those in the CDI group (Fig. 5c). In addition, the LEfSe analysis based on Linear discriminant analysis (LDA) score ≥ 4 showed that potential harmful bacteria such as C. difficile, E. coli, Mammaliicoccus sciuri which were abundant in the CDI group were much reduced in the endolysin treatment group. Thus, treatment with the endolysin CHAPSAP26-161 may help restore the gut microbiota to a healthy balance by suppressing pathogenic microbes, including C. difficile and those associated with C. difficile. Moreover, endolysin treatment increased the proportions of Muribaculaceae, Akkermansiaceae, Lachnospiraceae, Ruminococcaceae, and Lactobacillaceae (Fig. 5b). Therefore, endolysin CHAPSAP26-161 therapy could stabilize key microbial community structures associated with a healthy gut (Fig. 5).
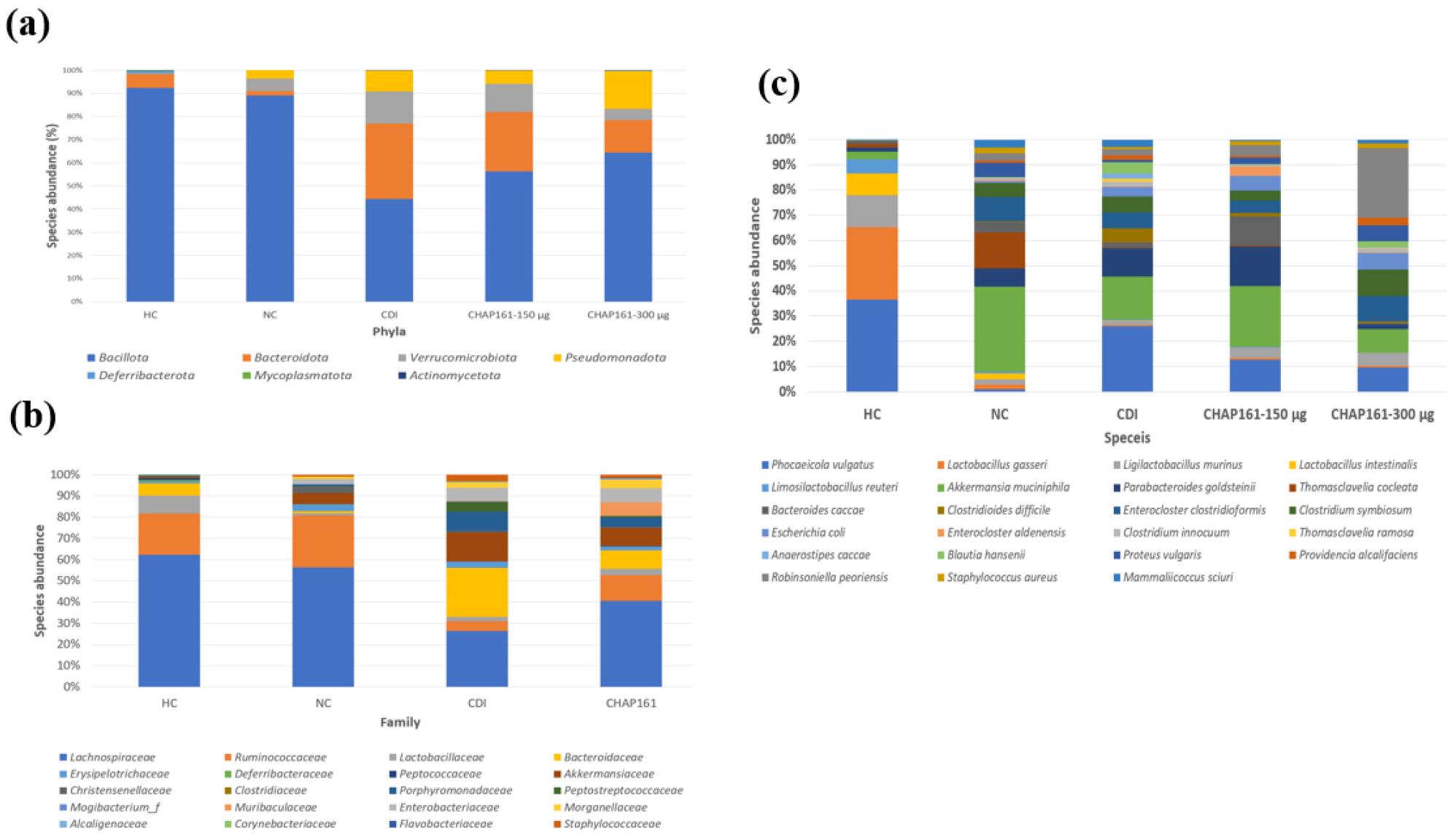
Fig. 5
Gut microbial composition of CDI murine model and endolysin-treated groups. (A) Gut microbial proportions at the phylum level; (B) gut microbial proportions at the family level; and (C) gut microbial proportions at the species level. HC, healthy control group; NC, negative control group; CDI, C. difficile infection group, CHAP161-150; CHAPSAP26-161 150 µg/mL, CHAP161-300; CHAPSAP26-161 300 µg/mL, CHAP161; total CHAPSAP26-161 Treatment Group.
DISCUSSION
CDI is a major public health problem worldwide and is commonly treated by antibiotics. Although FMT is gradually replacing antibiotic therapy, vancomycin remains the first treatment choice for CDI (34). However, antibiotics used against CDI may increase the chance of infection recurrence (34). In addition, transfer of the antibiotic-resistant microorganisms caused by FMT results in the deaths caused by the transplant (35). Therefore, treatment with bacteriophages that eliminate specific C. difficile strains is crucial for individuals with CDI as either primary or adjunctive treatments, considering the safety and efficacy of endolysin (15, 16, 36). The present study’s experimental results highlight that the endolysin CHAPSAP26-161 is a promising therapeutic agent against CDI. The multifaceted approach, encompassing gene cloning, protein expression and purification, antimicrobial activity assessment, toxigenic and nontoxigenic isolate characterization, cytotoxicity evaluation, and in vivo therapeutic effects, provides a comprehensive understanding of CHAPSAP26-161’s properties. The observed MIC and MBC values of 50 µg/mL for CHAPSAP26-161 against C. difficile strains underscore the potent antimicrobial efficacy of this endolysin (Table 1). Notably, the equal MIC and MBC concentrations for CHAPSAP26-161 and LysSAP26 indicate a consistent and robust antibacterial effect across different strains (Table 1). Nontoxigenic isolate (CD-M-5) identification through comprehensive molecular and immunogenic assays further emphasizes the potential clinical relevance of CHAPSAP26-161, particularly in treating infections caused by toxin-producing strains. The in vitro evaluation of CHAPSAP26-161 on A549 human cells revealed no cytotoxic effects at concentrations up to 750 µg/mL, indicating a favorable safety profile (37). At 1,000 µg/mL, cell viability slightly decreased, warranting further investigation to determine the upper limits of CHAPSAP26-161 concentration for safe therapeutic use. Nevertheless, the overall noncytotoxic nature of CHAPSAP26-161 at clinically relevant concentrations (≤750 µg/mL) is encouraging for its potential application as a therapeutic agent. In vivo experiments in a CDI murine model demonstrated significant positive outcomes following CHAPSAP26-161 treatment. The marked improvements in survival rates, body weight loss mitigation, diarrhea alleviation, and colon length recovery indicate the therapeutic potential of CHAPSAP26-161 (Fig. 3). Immunohistological staining further supported these findings by revealing a reduction in histological injuries, highlighting the protective effect of CHAPSAP26-161 against CDI-induced damage (Fig. 4).
Furthermore, 16S rRNA sequencing showed a marked decrease in the diversity and proportion of beneficial bacterial species, with a concurrent increase in pathogenic bacteria. Dysbiosis induced by C. difficile, characterized by a decrease in beneficial bacteria and an increase in pathogenic strains, was effectively addressed by CHAPSAP26-161 treatment. Following endolysin therapy, Muribaculaceae (can utilize mucin monosacharides), Bacteroidaceae, and Akkermansiaceae (potential probiotics) were restored to some extent (Fig. 5) (38, 39).
This study establishes a solid foundation for further exploration of CHAPSAP26-161 as an alternative therapy for CDI. However, notable constraints within our research were realized. One particularly significant limitation is the small number of mice utilized, constraining our ability to fully comprehend gut homeostasis after CHAPSAP26-161 therapy. Despite promising in vitro studies on CHAPSAP26-161, further research is necessary to fully grasp its potential as a therapeutic agent for CDI. Comprehensive preclinical assessments, which encompass studies on pharmacokinetics, and dosing optimization are indispensable to successfully translate CHAPSAP26-161 into clinical trials. Furthermore, with the emergence of new C. difficile variants and strains, continual surveillance and the development of effective therapies are needed. Prospective research avenues may involve elucidating the precise mechanism of action of CHAPSAP26-161, evaluating its efficacy against a broader spectrum of C. difficile strains, and conducting additional safety investigations.
CONCLUSION
The experimental results showed that CHAPSAP26-161 holds promise as an effective and safe therapeutic agent against CDI. The combined in vitro and in vivo findings provide a comprehensive understanding of its antimicrobial activity, safety profile, and therapeutic potential. Most importantly, CHAPSAP26-161 therapy reduces CDI-induced clinical outcomes and colon injury in mice. Microbial diversity restoration, decrease in pathogenic bacterial proportion, and increase in beneficial bacterial abundance highlight the potential of CHAPSAP26-161 not only in addressing CDI but also in mitigating CDI-associated dysbiosis. Additionally, the promising results in antimicrobial and gut microbiota homeostasis aspects pave the way for the potential clinical applications of CHAPSAP26-161 as a multifaceted therapeutic agent for CDIs.
AUTHOR CONTRIVUTIONS
Conceptualization, JK and SK; Formal analysis R.H.D and Y-JC; Funding acquisition, JK; Investigation, R.H.D, SK and Y-JC; Methodology, R.H.D and Y-JC; Project administration, JK and SK; Resources, JK; Software, R.H.D and Y-JC; Supervision, R.H.D, SK; Validation, JK and R.H.D; Visualization R.H.D and SK; Roles/Writing - original draft, R.H.D; and Writing - review & editing, R.H.D. Note that not all roles may apply to every manuscript, and authors may have contributed through multiple roles.